Many have pointed out that the neuronal dynamics in the hippocampus or entorhinal cortex enabling spatial navigation, may also facilitate ‘navigation through time’, into the past and future[1]–[4]. I am making a similar claim for the posterior cingulate cortex (PCC). When not solely relying on path integration, some animals use several landmarks sequentially to navigate to a goal. My claim is that when they do so, they simulate landmarks they can’t reach immediately to inform which closer landmark to move next to. In other words, they mentally simulate temporally distant landmarks to inform navigation decisions about temporally closer landmarks. The PCC originally evolved to do this but with time became a representational hub that simulates and integrates temporally distant memories, such as autobiographical memories. Once an organism’s generative model simulates temporally distant memories and finds itself returning to the same states across this temporal span, it may develop a form of self-referential self-awareness.
The article is structured as follows. In part 1, I discuss some background ideas about evolutionary continuity, constructive episodic simulation (CES), replay in rodents, and the role of the retrosplenial cortex (RSC) in ‘anchoring’ landmarks. Part 2 is an overview of the PCC's anatomy, functional connectivity and proposed functions. Part 3 introduces claim 1, connecting the idea of temporally distant landmarks to temporally distant memories, including autobiographical ones. Part 4 introduces claim 2, connecting the idea of temporally distant memories to self-awareness. I use research in developmental psychology and a Karl Friston quote to illustrate this link. Next, this is elaborated with Georg Northoff’s idea of temporal integration. Both claims 1 and 2 implicate the PCC and are followed by some supporting evidence from neuroimaging studies. Part 5 discusses two potential future research avenues, which I would love to explore during my Master’s and PhD. (1) ‘comparative corvid connectomics’ and (2) the interaction between replay bursts and default mode network activation.
Part 1
Neuroscience needs evolution[5]. Most neuroscientists would agree that many neuronal circuits are conserved across our evolutionary history, thus studying feedback control, explore-exploit dynamics and navigation in ancient mobile animals to primates is clearly productive[6], [7]. Yet, arguing for continuity in the evolution of mental phenomena is much more controversial[8]–[11].
One potentially evolutionary conserved mental phenomenon is constructive episodic simulation (CES)[12]? CES can broadly be understood as retrieving memories of past events and re-experiencing them, as if they are happening now, or imagining the future. Murray et al.[13] highlight that there are many terms in the literature that roughly translate to this; mental time travel, mental trial and error, scenario construction, prospection, autobiographical simulation, social modelling, and foresight. What connects all these things? Remembering the past and imagining the future activates the same brain regions, including the medial prefrontal cortex (mPFC), posterior cingulate cortex (PCC), retrosplenial cortex (RSC), and the hippocampal formation[12], [14]–[16]. This suggests that a unified neuronal mechanism may underly the episodic construction of the past and future. From now on, we will call this mechanism CES. For a visual illustration of CES, see Fig. 1 below.
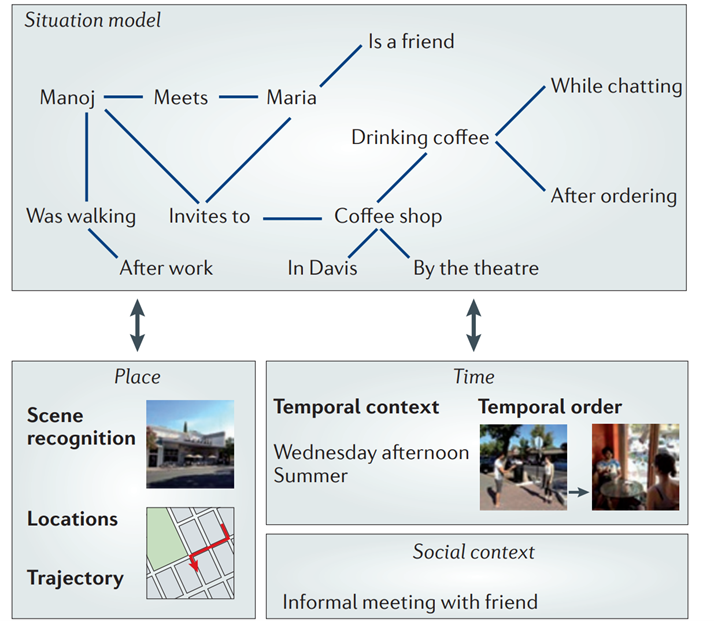
Fig. 1: Graphical illustration for constructive episodic simulation (CES). The image was taken from Fig. 3 in REF[17]. Many thanks to Charan Ranganath for letting me use it. In the original publication, this illustration was used to explain the role of the posterior medial (PM) system as facilitating the encoding and retrieval of an event’s context in a modality-independent way. The PM system consists of the parahippocampal cortex, retrosplenial cortex (RSC), posterior cingulate (PCC), angular gyrus, precuneus (Prec), anterior thalamus and mammillary bodies, and medial prefrontal cortex (mPFC) [17], [18].
If we want to return to the idea of evolutionary continuity, we should probably ask: What are ecologically valid examples of CES in non-human animals (henceforth animals)? One intriguing finding is that in spatial navigation experiments with rodents, they sometimes suddenly stop moving at choice points as if they are deliberating where to go next. For example, in a T-maze set-up, they might stop and ‘ponder’ whether to turn left or right. In electrophysiological studies recording the hippocampal place cells of freely moving mice, it was found that when the mice were approaching the T-choice point, place cells encoding the location ahead (left or right of the choice point) were already firing before the mouse was there. Since the frequencies of these place cells firing are in a theta cycle, this is known as the theta rhythm. And known as sharp-wave ripples, when mice are at rest or sleeping, there is high-frequency firing of place cells, which encode non-local path trajectories[19]. Commonly, known as ‘replay’, these findings are clearly suggestive of rodents mentally simulating previously visited places in order to inform spatial navigation decision-making.
Other research on spatial navigation highlights the role of landmarks. Lesion, electrophysiological and fMRI studies in humans, rodents, and monkeys have implicated the retrosplenial cortex (RSC) in landmark use for orientation[20]–[24]. Although other regions are also involved in the visual recognition and perceptual analysis of landmarks, it has been suggested the role of the RSC is to ‘anchor’ landmarks to the cognitive map produced by the hippocampus and entorhinal cortex[21]. This anchoring might involve processes such as the integration of allocentric-egocentric information[25], [26] and directional heading, as encoded by head direction cells[27].
Interestingly, a lot of current research explores whether the cognitive map metaphor could also be applied to non-spatial maps such as conceptual or social spaces[21], [28]–[32]. As a thought experiment, if we apply the same logic to the RSC – see also REF[33], we could argue that it might be involved in anchoring non-spatial landmarks. Going back to our T-maze choice point example, we could think of this choice point as a landmark (Fig. 2a), and transform this into an episodic landmark (Fig. 2b). Clearly, the world is more complex than an episodic T-maze with events leading to other binary episodic outcomes. Yet, we could imagine scaling up the complexity by thinking of it in terms of a large episodic cognitive map (like a multidimensional network), where each landmark node represents an event that has contingent outcomes, which might lead to temporally subsequent landmark nodes themselves.
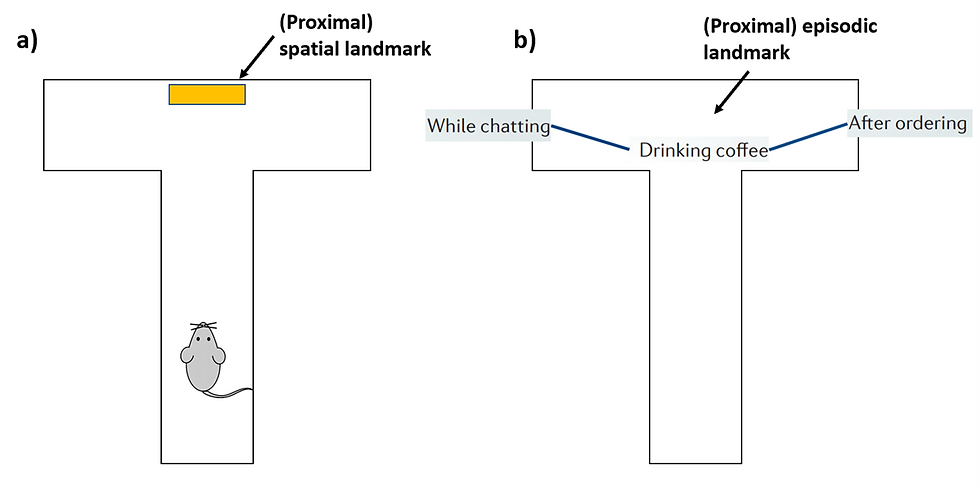
Fig. 2. a) Classical T-maze, with a proximal spatial mark. b) Episodic T-maze, with a proximal episodic landmark (derived from Fig. 1) It is worth highlighting that in a), there is a temporal precedence relationship. Only after reaching the spatial landmark, the mouse may move left or right. Similarly, in b) the episodic event of drinking coffee ‘while chatting’ or ‘after ordering’ are logically contingent on ‘drinking coffee’. Thus, the temporal hierarchy in a) was translated into a conditional hierarchy in b).
For the sake of simplicity, let’s return to the spatial case. Instead of a T-maze (Fig. 2a), what would be a more ecologically realistic environment for a mouse? To recreate one, we might have to build an arena with choice points with multiple paths, different levels, facilitating horizontal and vertical travel, a combination of proximal and distal landmarks, and so forth. Considering all this, the mouse would not only have to make sense of the spatial complexity of this cognitive map but also the temporal complexity of navigating it. Since it may not solely rely on path integration and might not see a distal landmark close to its goal, it would have to remember, which landmarks can guide it to which other landmarks. Therefore, the mouse would have to know at which landmark to turn left, right, etc. Depending on its planned trajectory, if landmarks are more temporally distant, it has to simulate sequentially more landmark memories. For a computational model of this idea, using an attractor network, see REF[34].
Is this complexity reflected in brain anatomy? In proportion to their overall brain size, mice have a very large RSC[35]. This suggests the importance of landmark-use in their ecology or a more general function of their RSC as an integration hub for navigation information[36]. In human and non-human primates, the retrosplenial cortex is with respect to overall brain size much smaller[35]. This may seem surprising considering the complexity of foraging techniques, large home ranges, and frequent use of landmarks across various primate species[37]. One explanation would be that primate intelligence and brain expansion are mostly explained by the social hypothesis and very little by ecological factors, thus leading to disproportionately less growth in brain regions specialized in spatial navigation. This is not a common view[38], [39]; think about the ability of orangutans to find and feed on food sources, which vary spatially and temporally depending on the seasons, the expiry dates of different fruits, etc[40]. And clearly, social and foraging hypotheses are co-dependent, especially in primate fission-fusion societies, where individuals need increased capacity to monitor across time and space the comings and goings of other group members, and how this influences their foraging strategies[41].
Another possible explanation is that in the evolutionary lineage between rodents and primates, cortical expansion created a new functionally heterogeneous region, also supporting landmark use and complex-long distance spatial navigation. One good candidate for such a region is the posterior cingulate cortex (PCC). It is anatomically close to the RSC (see Fig. 3), and rodents don’t have a homologous region for it.

Fig. 3 Coronal view of the left hemisphere of a modified Brodman human adult reference atlas. Image 72 from 106, thus roughly 2/3rd towards the posterior end. (Note: The choice of image 72 was relatively arbitrary. For different images/slices the relative size of the regions may vary. Feel free to play around with this here).Magnified image shows dorsal posterior cingulate cortex (dPCC) (Brodmann Area 31), ventral posterior cingulate cortex (vACC) (A23), retrosplenial cortex (RSC) (A29, A30), and hippocampal formation (HF). Screenshot taken from the Allen Brain Reference Atlas[42].
Part 2
Before, we discuss the PCC with respect to the previously discussed, a general background overview of this region may be useful. (Click here to skip for a summary of Part 2). It is one of the main regions in the default mode network (DMN). The phylogenetic origins of the PCC (at least to my knowledge) are unclear. When comparing the laminar proportions of prosimians and anthropoids relative to brain size, for both the ventral and dorsal PCC (Brodmann A23, A31), the anthropoids have relatively larger outer main laminae and granular layers. This may have enabled higher integration and differentiation of incoming information in the PCC of anthropoids, potentially leading to adaptation in the prosimians-anthropoid split[43]. Relatedly, a very recent study also discovered a new sulcus in the PCC, called the inframarginal sulcus (ifrms). Willbrand et al.[44] call it a tripartite landmark because it is A) cortically thick, B) in a lightly myelinated portion of PCC, and C) is functionally also connected to the lateral frontoparietal network (besides being a major node in the DMN). Comparing morphological differences across humans and chimpanzees, they found that ifrms was cortically thinner in chimpanzees, potentially elucidating an evolutionarily recent adaptation.
The overall function of the PCC is not well understood. Activity in PCCs has been associated with mind-wandering[45], retrieval of autobiographical memories[46], emotional words[47], self-referential stimuli[48], [49], and reduced glucose metabolic activity was associated with disorientation for time and place in Alzheimer’s patients[50]. For more proposed functions see also (REF[51] Table 1). Yet, there is no convincing story for the overall function of the PCC. One study using real-time fMRI neurofeedback in meditators, proposed that the self-referential process of “getting caught up in” one’s experience may be this overall function[52]. Pearson et al.[51] argued for the PCC’s role in environmental change detection, facilitating mental simulation of different scenarios and the rapid switching across behavioural policies. They also suggest the PCC’s more active role towards the external environment. Supporting this, whilst metabolic activity in the DMN decreases during task performance, this is less the case for the PCC [and the precuneus][53]. Pearson et al.[51] illustrate their proposal using experimental work with macaques, thus suggesting evolutionary continuity for the PCC’s role in cognition.
Clearly, thinking about a brain region’s function as an isolated area is also not fruitful in neuroscience. In graph theory, a provincial hub is a high-degree node that connects many nodes within a network, whilst a connector hub is a high-degree node that connects different segregated modular networks [54]. The PCC may be considered both. The ventral part of the PCC (Fig. 3) is a provincial hub that shows a strongly correlated activity with the rest of the DMN, particularly with the mPFC (see REF[55] Fig. 8; REF[56] Fig. 2a). The functional connectivity from this provincial hub in the ventral PCC is quite a dominant pattern, thus may hide the more subtle functional integration of the dorsal PCC (Fig. 3) with brain regions outside the DMN. Using a temporal concatenation group independent component analysis, Leech et al.[56] extracted 10 independent components from resting-state fMRI data with the dorsal PCC as a seed. From these 10 regions connected to the dorsal PCC, 5 subregions included the left- and right-lateralized frontoparietal networks covering inferior parietal and distributed frontal regions, a motor network, a sensory network, and a more executive network. Since these 5 subregions are not typically associated, this supports the dorsal PCCs’ role as a connector hub. Supporting this, a study using fMRI, diffusion spectrum imaging, and graph-theoretical measures such as centrality and efficiency also identified the PCC [and the precuneus] as brain-wide connector hubs[57].
Which functional connections from/to the PCC are relevant for CES? A phenomenal study by Smallwood et al.[58] may allow us to disentangle this. The study involved resting-state fMRI scans on Day 1 and multi-dimensional experience sampling (MDES) on Day 2. The latter involved unexpectedly interrupting participants during an easy laboratory task to answer questions about the contents of their experience immediately before the interruption. This way the researchers could quantify individual differences in mental content through dimensions such as past-future, self-other, level of detail or modality. They found a lot of similarity in content for reports of past and future thinking, thus combined this into the single ‘CES-like’ measure mental time travel. Comparing the cross-sectional variance between MDES scores and functional connectivity of the DMN, they found that increased coupling between the temporal lobe and the PCC was predictive of mental time travel. Smallwood et al.[58] conclude that the PCC may “function as a representational hub, enabling it to integrate information from different cortical regions that provide the basis of the experiential content to which we attend when we transcend the here and now using imagination”.
Okay, let’s summarize all of this. Although the phylogenetic origin of the PCC is unclear, there is some evidence to suggest that after the prosimians-anthropoid split and the pan-homo split, this region experienced important anatomical changes. The ventral and dorsal PCC are provincial and connector hubs respectively, with functional connections to many brain regions, including the DMN and temporal lobe. The region is involved in self-referential processing, autobiographical memories, mental simulation, and orientation in space and time, yet there is no consensus on overarching functional specialisation.
Part 3
Claim 1: The overarching function of the posterior cingulate cortex (PCC), as a central hub within many networks, is to simulate and integrate temporally distant episodic memories. These memories may have been ‘anchored’ in the retrosplenial cortex (RSC). Originally, this evolved in an ancestral mammal to simulate temporally distant landmarks, and integrate this information into planning strategies. Throughout evolution, this became a domain-general function that allowed simulating and integrating temporally distant episodic memories. In humans, and maybe other animals, this now facilitates autobiographical memories, which are essentially memories nested across long timescales; years, months, weeks, days and socially constructed time periods (i.e. school time, adulthood).
Is there evidence to support this? Yes! One interesting finding in the cognitive map literature highlights the role of ‘distance’ in the dorsal PCC. Tavares et al.[32] suggest that the brain may make use of cognitive maps to represent not just physical space but also ‘social space’. They explored this with a virtual role-playing game paradigm, where participants had to ‘navigate’ a social space by interacting with six characters. Tavares et al.[32] found that the fMRI response in the hippocampus scaled with the angle of the vector from the participant’s position to the character’s position in the social space, with the greatest response to characters with higher power and high affiliation. On the contrary, dorsal PCC fMRI activity scaled with the magnitude of the vector, with the greatest response to more socially distant characters. Thus, although characters with higher power and higher affiliation are ecologically more likely to be relevant for self-related processing, the PCC actively encodes larger distances in social space.
Let’s further consider the idea of temporal distance through the example of language. Language is interpreted across multiple timescales (from phonemes to words, to sentences and narratives). Using this intuition, Hasson et al.[59] suggest that ‘process memory’ for sensory input in shorter timescales (i.e., integrating a few phonemes to detect a word) may be localised in sensory regions such as the primary auditory cortex. Process memory for longer timescales (i.e., integrating sentences over time while comprehending a narrative) requires high-order regions such as the medial temporal lobe (MTL). The proposal is that these regions have different ‘temporal receptive windows’ (TRWs). Hasson et al.[59] tested this proposal using fMRI and electrocorticography respectively in an audio narrative and audio-visual movie paradigm. In both paradigms, the stimuli across temporal scales were scrambled. For example, in the fMRI and audio narrative paradigm, participants listened to stories where word-order, sentence-order, or paragraph-order were scrambled. They found that the BOLD response was comparable across scrambled and non-scrambled conditions in the early auditory areas. Going up the processing hierarchy, the BOLD response was increasingly affected by the stimulus history. Since word-order scrambling vs sentence-order scrambling corresponded to specific timescales in the sensory input. they could map TRWs to cortical regions depending on their differential BOLD response across scrambled vs non-scrambled stimuli. Hasson et al.[59] highlight that MTL areas, with the highest TRWs, broadly overlap with the DMN, including the PCC.
What about the RSC? In the spatial navigation domain, this region anchors landmarks onto a cognitive map. Could it have a similar anchoring mechanism for temporally distant autobiographical memories? This intuition is supported by the finding that patients with RSC damage show retrograde amnesia for autobiographical events[20], [60]. Relatedly, one study found that RSC activity scaled with the degree of subjective ‘reliving’ during autobiographical memory retrieval[61].
Let’s stick with the topic of temporally distant memories but relate it to the self.
Part 4
Claim 2: Once an organism is able to simulate temporally close and distant memories, it may recognize itself as a common denominator across those simulations. Thus, the ability to simulate and integrate temporally distant memories may significantly contribute to the emergence of self-awareness. Furthermore, once an organism is self-aware (to various levels), it may self-project itself into mental simulations, thus experiencing them from an [embodied] first-person perspective. This perspective may facilitate the simulation of temporally distant memories in a more integrated and flexible way. These two processes may have a reciprocal evolutionary relationship with a potential locus in the posterior cingulate cortex (PCC).
Note, that A) I am not insisting that simulating temporally distant memories is the only precondition for the emergence of self-awareness, there may be other processed involved[62], [63]. And B) I am not saying the PCC has a monopoly on self-referential processing, other regions such as the medial prefrontal cortex (mPFC) may also be very important[64], [65], our coupling between these two regions[58], [66].
Before we turn to neuroscientific work connecting temporally distant memories with the self, let’s consider some theoretical and developmental intuitions about the self. Some authors suggest that self-awareness is necessary for CES, as an autonoetic consciousness[67] or self-schema[16] is required to project one’s [embodied] self into the mental simulation[68]. This resembles the second half of claim 2 about self-projection.
The idea that self-awareness precedes the development of CES is also loosely supported by findings in developmental psychology. Human infants can pass the mirror-mark test during their second year [69], [70]. however only 12 or 24 months later, they can pass a live or delayed video version of the mark test[71]; it seems that only by the age of four, their self-concept extends through time and space. At the same age, children are also able to entertain the idea of the future playing out in different scenarios. For example, in one experimental set-up, a reward was dropped into a vertical tube with two exits at the bottom, “like an upside-down Y”. In preparation for the drop, two-year-old children would typically only cover one or the other exit with their hands, whilst four-year-old children would consistently cover both exits[72]. To highlight a point about mental evolutionary continuity, chimpanzees have also passed a delayed video version of the mirror-mark test[73].
To elucidate the first half of claim 2, where temporally distant memories evolutionarily precede the emergence of self, Karl Friston’s idea of ‘temporal thickness’ might be useful[62]. Once, a system has internal dynamics that allow it to make sense of a past and future, and if it acts upon this information in a systematic way (i.e. executing planned behaviour), it may notice that a common denominator within that past and future is itself. He also illustrates this using a dynamical systems approach:
Heuristically, the difference between thick and thin models is manifest in terms of the structure of the random dynamical attractor. A key aspect of this attracting manifold is the time elapsed between revisiting the same state (or neighborhood).(…) I revisit the same states over very long time periods (…), for example, every morning I take my latte in the park outside my office—and every Christmas I attend midnight mass” [62]
In short, self-organisation is defined by a generative model that spans across temporal contexts but revisits the same [or nearby] states.
In claims 1 and 2, I also suggest the PCC’s function involves not just simulating but integrating information from temporally distant memories. I will unpack this using Georg Northoff’s idea of ‘temporal integration’[74]–[76]. It suggests that the temporal continuity we experience on the phenomenological level is mediated by the self, which integrates different timescales. This proposal conceives of the integration of various selves, where an interoceptive self, an [predictive] extero-proprioceptive self, and a self-referential self – working across increasingly longer timescales – are nested in a self-hierarchy[77]–[79]. For an overview of which brain regions are involved in different selves (see Fig. 4). The proposal of a nested-integrated self also echoes the work of Sui & Humphreys[80], who framed the self as an ‘integrative glue’ that binds perceptual, memory, and decision-making to an information-sparse self-referential schema.
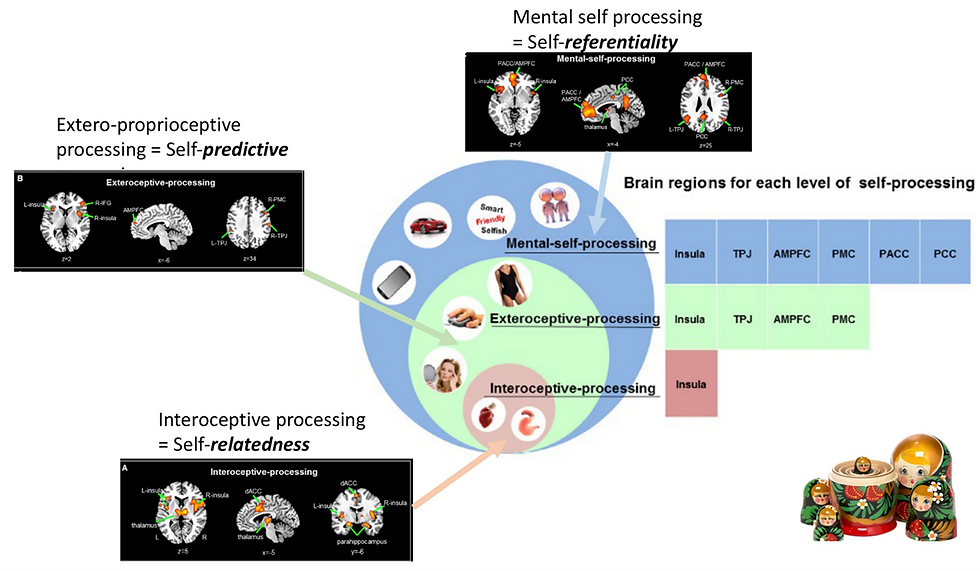
Fig. 4 Illustration of interoceptive self, extero-proprioceptive self, and self-referential as nested (like a Russian doll). Within this nested self-hierarchy, brain regions specific to lower selves are nested within higher selves. PACC and PCC are specific to self-referential self. Abbreviations: Insula, temporal parietal junction (TPJ), anteromedial prefrontal cortex (AMPFC), posterior medial cortex (PMC), pregenual anterior cingulate cortex (PACC), posterior cingulate cortex (PCC). Image is taken from Figure 4 in REF[78] – brain regions identified in REF[79]. Many thanks to Georg Northoff for letting me use it.
Within this nested temporally integrating self-hierarchy, is the PCC really at the top? Kolvoort et al.[74] suggest that dynamics such as the cross-frequency coupling, autocorrelation window (ACW), or power law exponent (PLE) may be indicative of temporal integration on the neuronal level. That is the integration of neuronal oscillations on different frequencies. They explore this using the well-established perceptual matching task[81], in which participants are assigned geometric shapes with the labels ‘self’, ‘friend’, and ‘other’ and need to match these geometric shapes to the subsequently presented labels. The self-prioritization effect (SPE)[80] finds that participants show efficient processing for self-related stimuli, measured as increased accuracy or decreased response time. Kolvoort et al.[74] modify the perceptual matching task by introducing varying delays (40, 120 or 700ms) between the presentation of the shape and label. They compare the SPE across different delay conditions (see Fig. 5). As you can see, there was a steep positive slope observed for no delay. This is indicative of individual differences where high SPE correlates with higher accuracy. Since this effect did not carry over for the time-delayed conditions, it seems the SPE changes with delays. They observed that for longer delays, individuals’ SPE positively correlated with higher PLE and longer ACW. This suggests that interindividual differences in temporal integration on the neuronal level, as measured through resting-state PLE and ACW, may explain the interindividual changes of the SPE due to temporal delays on the psychological level. Kolvoort et al.[74] found this effect to be stronger in the PCC and pregenual anterior cingulate cortex (ACC) compared to the visual and motor cortex.

Fig. 5. On x-axis is the probability of correct answers due to self-prioritization. On the y-axis are subjects ordered by no delay Self-Prioritization Effect (SPE) score. The positive steep curve in no delay condition suggests that higher SPE scores correlate with higher accuracy. Findings from Fig. 4a in REF[74]. Many thanks to Ivar Kolvoort and Georg Northoff for letting me use it.
Let’s return to Georg Northoff’s different selves[77], [78]. In Fig. 4, PCC-mediated long timescales may map onto the self-referential self but not to intero-/exteroceptive selves. Returning to the meditation study, which found that meditators could reduce PCC activity by focussing their attention on their breathing[52], we may argue that their interoceptive self, keeping track of their breathing, was limited to a timescale of seconds. Relatedly, Saxe and Powell[82] found that DMN regions including the PCC did not differentially active to stories about people’s bodily sensations but did so for stories conceiving other people’s thoughts. To reiterate, different selves work on different timescales. Linking this back to evolutionary continuity, we may be sceptical of attributing autobiographical memories or self-referential processing to non-human/ non-great ape species. Yet, we may be more open to attributing interoceptive selves to various species, yes, they might be self-aware of their heartbeat. And going back to mice simulating landmarks, we could imagine them having [predictive] extero-proprioceptive selves, thus being self-aware of their head direction and self-motion[83] – see also the embodied self[84], [85]. Well, once we accept these claims, we may be more open consider the evolution of a self-referential self, with a locus in the PCC, to begin somewhere before the pan-homo split, whether it is within the primate lineage or much earlier.
Part 5
Claims (or hypotheses) 1 and 2 are pretty bold. How could one go about empirically testing them? I propose 2 research avenues, which I would like to explore during my Master's and PhD. (1) A ‘comparative corvid connectomics’ project investigating whether anaesthetized corvids would have something homologous to the mammalian default mode network (DMN) with a posterior cingulate cortex (PCC) homologue being a major functional hub. And explore whether variation in network typology of such functional connectomes could be explained by behavioural markers and phylogenetic distance across evolutionary lineages. (2) Exploring the intersection of resting-state networks, such as the DMN, with the dynamics of hippocampal neural ensembles underlying replay.
(1) For a theoretical overview of comparative connectomics see REF[86], [87], and for two applications with primates and mammals see REF[88], [89].
But besides ‘comparative corvid connectomics’ sounding really cool, why should we do it? Birds don’t have a PCC or neocortex, but instead a very large pallium[90], [91]. Yet, many bird species show very sophisticated and flexible cognitive abilities, prompting questions about parallel evolution and homologies in mammalian and avian brains[92]- [96]. Particularly, corvids have shown impressive constructive episodic simulation (CES) behaviours like mental time travel and theory of mind, comparable to the level of great apes[97]–[99]. For example, after Western scrub jays cache (hide) perishable food such as wax worms in specific sites, they preferentially return to these sites after short periods of time but not after long time intervals, as they know the worm has already decayed. This suggests they have information about the what, where and when they cached something[100]. And when western scrub jays notice that they are observed by other birds, they tend to re-cache their food more often[101]. This only happens if these jays have experience of stealing food themselves[102]; “it takes a thief to know one”. There is also evidence for mirror-self recognition in Eurasian magpies[103], although this hasn’t been successfully replicated with magpies or other corvids[104]–[107], and there are many methodological open questions[108], [109].
Is there a PCC homologue in birds? Anatomical work with pigeons has suggested the corticoidea dorsolateralis as being homologous to the mammalian cingulate cortex[110]. One structural connectomics study on pigeons found that this region was one of the major hubs in terms of anatomical connections[111]. But, since the last common ancestor of pigeons and corvids lived in the paleocene epoch, so more than 56million years ago[112], it’s unclear whether this is also true for corvids. Furthermore, we don’t know anything about the region’s functional connectivity.
What would a functional corvid connectomics approach look like? fMRI experiments with mice, rats, cats and monkeys under anaesthesia have found a DMN-homologous network in these animals[113]–[116]. This suggests that even under anaesthesia, we can still learn about this resting-state network – see also REF[117], [118]. Thus, one research question could ask whether the functional network typology of anaesthetized corvids is similar to the mammalian DMN. Although neuroanatomical grounding will surely be important, parcellation of the corvid fMRI data could also be achieved using an independent component analysis[119], [120].
A key component to comparative corvid connectomics, will be using graph-theoretic measures[54], [121]–[124] to compare functional network properties across species. There are open questions about which species to compare. Comparing corvid and primates connectomes clearly makes sense because there is already data on the latter, and both groups exhibit similar levels of CES-like behaviours. On the other hand, when studying connectomes intra-family (Corvidae) or intra-order (Passeriformes), variation in network typologies can be much better compared with phylogenetic distance across those species, giving us indications about the evolutionary direction of network changes.
(2) One study managed to show that reply bursts in humans coincided with alpha waves in the posterior part of the DMN, including the PCC[125]. The key was to study replay and DMN, not with fMRI but MEG, which has a higher temporal resolution. This meant that the onset of high-frequency sharp wave ripples could be detected and compared to the onset of transient DMN activations on the millisecond scale. One suggestion in the paper is that the strong alpha activity in the DMN may inhibit bottom-up sensory perception during inward-oriented attention, thus preventing interference from sensory inputs, and making sure replay is ‘played out’. This raises an interesting evolutionary point about the potential necessity of long temporal dynamics for internally directed cognition. Brains of species that are unable to produce long timescale dynamics, such as low-frequency alpha oscillations, might be unable to suppress sensory input long enough, for mental simulations to emerge longer than just transiently.
This notion also echoes James McClelland et al.[126] complementary learning systems theory of the hippocampus and neocortex, which they developed based on insights from connectionist (deep learning) models. Memories of experiences are first stored in the hippocampal system via synaptic changes. The neocortex (like a deep learning model) then learns about the structure of these ensemble experiences by changing its ‘connections’. Since this type of learning is slow, the neocortex can create a stable generative model of the world. On the other hand, the hippocampus is a rapid sequence generator, that continuously creates new memories, which in turn slowly update the generative model of the neocortex. Thus, the neocortex work on long timescales, whilst the hippocampus does so on short timescales. How these dynamics interact on various timescales interact is a very important open question.
But what do replay bursts do? Some suggest they might implement compositional computation[127]. And how does the DMN integrate the information from the replay bursts? One conception of the DMN as a principal gradient of macroscale cortical organization[128] is adapted to include the hippocampus at the top of the gradient above the default mode network[129]. In this proposal, replay cascades move down along this gradient (like an avalanche). Hub regions in the DMN then exhibit ‘critical’ dynamics which regulate replay cascades so that most cascades have a high probability of being a very small avalanche spreading only very briefly across the gradient, whilst few very replay cascades have long-lasting effects all over the gradient. As a result, there is a power law distribution where very large events account for most of the temporal dynamics. My intuition is that the PCC will be an important region of interest to further explore these questions. I am excited.
References
[1] J. B. Issa, G. Tocker, M. E. Hasselmo, J. G. Heys, and D. A. Dombeck, ‘Navigating Through Time: A Spatial Navigation Perspective on How the Brain May Encode Time’, Annual Review of Neuroscience, vol. 43, no. 1, pp. 73–93, 2020, doi: 10.1146/annurev-neuro-101419-011117.
[2] G. Buzsáki and R. Llinás, ‘Space and time in the brain’, Science, vol. 358, no. 6362, pp. 482–485, Oct. 2017, doi: 10.1126/science.aan8869.
[3] G. Buzsáki and E. I. Moser, ‘Memory, navigation and theta rhythm in the hippocampal-entorhinal system’, Nat Neurosci, vol. 16, no. 2, Art. no. 2, Feb. 2013, doi: 10.1038/nn.3304.
[4] M. Jajayeri, Mehrdad, ‘Timing via counting using attractor networks in the entorhinal cortex’, presented at the Bernstein Conference 2022, 2022. doi: 10.12751/nncn.bc2022.005.
[5] P. Cisek and B. Y. Hayden, ‘Neuroscience needs evolution’, Philosophical Transactions of the Royal Society B: Biological Sciences, vol. 377, no. 1844, p. 20200518, Feb. 2022, doi: 10.1098/rstb.2020.0518.
[6] P. Cisek, ‘Evolution of behavioural control from chordates to primates’, Philosophical Transactions of the Royal Society B: Biological Sciences, vol. 377, no. 1844, p. 20200522, Feb. 2022, doi: 10.1098/rstb.2020.0522.
[7] G. Pezzulo and P. Cisek, ‘Navigating the Affordance Landscape: Feedback Control as a Process Model of Behavior and Cognition’, Trends in Cognitive Sciences, vol. 20, no. 6, pp. 414–424, Jun. 2016, doi: 10.1016/j.tics.2016.03.013.
[8] M. C. Corballis, ‘Mental time travel: a case for evolutionary continuity’, Trends in Cognitive Sciences, vol. 17, no. 1, pp. 5–6, Jan. 2013, doi: 10.1016/j.tics.2012.10.009.
[9] S. J. Shettleworth, ‘Clever animals and killjoy explanations in comparative psychology’, Trends in Cognitive Sciences, vol. 14, no. 11, pp. 477–481, Nov. 2010, doi: 10.1016/j.tics.2010.07.002.
[10] T. Suddendorf, ‘Mental time travel: continuities and discontinuities’, Trends in Cognitive Sciences, vol. 17, no. 4, pp. 151–152, Apr. 2013, doi: 10.1016/j.tics.2013.01.011.
[11] D. J. Povinelli and T. M. Preuss, ‘Theory of mind: evolutionary history of a cognitive specialization’, Trends in neurosciences, vol. 18, no. 9, Art. no. 9, 1995.
[12] D. R. Addis, A. T. Wong, and D. L. Schacter, ‘Remembering the past and imagining the future: Common and distinct neural substrates during event construction and elaboration’, Neuropsychologia, vol. 45, no. 7, pp. 1363–1377, Jan. 2007, doi: 10.1016/j.neuropsychologia.2006.10.016.
[13] E. A. Murray, S. P. Wise, and K. S. Graham, The evolution of memory systems: ancestors, anatomy, and adaptations, First Edition. Oxford, United Kingdom ; New York, NY: Oxford University Press, 2017.
[14] D. L. Schacter and D. R. Addis, ‘On the constructive episodic simulation of past and future events’, Behavioral and Brain Sciences, vol. 30, no. 3, pp. 331–332, Jun. 2007, doi: 10.1017/S0140525X07002178.
[15] D. L. Schacter, D. R. Addis, D. Hassabis, V. C. Martin, R. N. Spreng, and K. K. Szpunar, ‘The Future of Memory: Remembering, Imagining, and the Brain’, Neuron, vol. 76, no. 4, p. 10.1016/j.neuron.2012.11.001, Nov. 2012, doi: 10.1016/j.neuron.2012.11.001.
[16] D. R. Addis, ‘Mental Time Travel? A Neurocognitive Model of Event Simulation’, Rev.Phil.Psych., vol. 11, no. 2, pp. 233–259, Jun. 2020, doi: 10.1007/s13164-020-00470-0.
[17] C. Ranganath and M. Ritchey, ‘Two cortical systems for memory-guided behaviour’, Nat Rev Neurosci, vol. 13, no. 10, Art. no. 10, Oct. 2012, doi: 10.1038/nrn3338.
[18] M. Ritchey, L. A. Libby, and C. Ranganath, ‘Chapter 3 - Cortico-hippocampal systems involved in memory and cognition: the PMAT framework’, in Progress in Brain Research, vol. 219, S. O’Mara and M. Tsanov, Eds. Elsevier, 2015, pp. 45–64. doi: 10.1016/bs.pbr.2015.04.001.
[19] L. T. Hunt et al., ‘Formalizing planning and information search in naturalistic decision-making’, Nat Neurosci, vol. 24, no. 8, Art. no. 8, Aug. 2021, doi: 10.1038/s41593-021-00866-w.
[20] E. Maguire, ‘The retrosplenial contribution to human navigation: A review of lesion and neuroimaging findings’, Scandinavian Journal of Psychology, vol. 42, no. 3, pp. 225–238, 2001, doi: 10.1111/1467-9450.00233.
[21] R. A. Epstein, E. Z. Patai, J. B. Julian, and H. J. Spiers, ‘The cognitive map in humans: spatial navigation and beyond’, Nat Neurosci, vol. 20, no. 11, Art. no. 11, Nov. 2017, doi: 10.1038/nn.4656.
[22] A. S. Mitchell, R. Czajkowski, N. Zhang, K. Jeffery, and A. J. D. Nelson, ‘Retrosplenial cortex and its role in spatial cognition’, Brain and Neuroscience Advances, vol. 2, p. 2398212818757098, Jan. 2018, doi: 10.1177/2398212818757098.
[23] M. J. Buckley and A. S. Mitchell, ‘Retrosplenial Cortical Contributions to Anterograde and Retrograde Memory in the Monkey’, Cerebral Cortex, vol. 26, no. 6, pp. 2905–2918, Jun. 2016, doi: 10.1093/cercor/bhw054.
[24] S. D. Auger, S. L. Mullally, and E. A. Maguire, ‘Retrosplenial Cortex Codes for Permanent Landmarks’, PLOS ONE, vol. 7, no. 8, p. e43620, Aug. 2012, doi: 10.1371/journal.pone.0043620.
[25] B. J. Clark, C. M. Simmons, L. E. Berkowitz, and A. A. Wilber, ‘The retrosplenial-parietal network and reference frame coordination for spatial navigation’, Behavioral Neuroscience, vol. 132, pp. 416–429, 2018, doi: 10.1037/bne0000260.
[26] M. Stacho and D. Manahan-Vaughan, ‘Mechanistic flexibility of the retrosplenial cortex enables its contribution to spatial cognition’, Trends in Neurosciences, vol. 45, no. 4, pp. 284–296, Apr. 2022, doi: 10.1016/j.tins.2022.01.007.
[27] J. S. Taube, ‘Head direction cells and the neurophysiological basis for a sense of direction’, Progress in Neurobiology, vol. 55, no. 3, pp. 225–256, Jun. 1998, doi: 10.1016/S0301-0082(98)00004-5.
[28] A. O. Constantinescu, J. X. O’Reilly, and T. E. J. Behrens, ‘Organizing conceptual knowledge in humans with a gridlike code’, Science, vol. 352, no. 6292, pp. 1464–1468, Jun. 2016, doi: 10.1126/science.aaf0941.
[29] C. M. Wu, E. Schulz, M. M. Garvert, B. Meder, and N. W. Schuck, ‘Similarities and differences in spatial and non-spatial cognitive maps’, PLOS Computational Biology, vol. 16, no. 9, p. e1008149, Sep. 2020, doi: 10.1371/journal.pcbi.1008149.
[30] R. Bottini and C. F. Doeller, ‘Knowledge Across Reference Frames: Cognitive Maps and Image Spaces’, Trends in Cognitive Sciences, vol. 24, no. 8, pp. 606–619, Aug. 2020, doi: 10.1016/j.tics.2020.05.008.
[31] T. E. J. Behrens et al., ‘What Is a Cognitive Map? Organizing Knowledge for Flexible Behavior’, Neuron, vol. 100, no. 2, pp. 490–509, Oct. 2018, doi: 10.1016/j.neuron.2018.10.002.
[32] R. M. Tavares et al., ‘A map for social navigation in the human brain’, Neuron, vol. 87, no. 1, Art. no. 1, 2015.
[33] R. G. Munn and L. M. Giocomo, ‘Multiple head direction signals within entorhinal cortex: origin and function’, Current Opinion in Neurobiology, vol. 64, pp. 32–40, Oct. 2020, doi: 10.1016/j.conb.2020.01.015.
[34] E. T. Rolls, ‘Neurons including hippocampal spatial view cells, and navigation in primates including humans’, Hippocampus, vol. 31, no. 6, pp. 593–611, 2021, doi: 10.1002/hipo.23324.
[35] F. Burles, A. Umiltá, L. H. McFarlane, K. Potocki, and G. Iaria, ‘Ventral—Dorsal Functional Contribution of the Posterior Cingulate Cortex in Human Spatial Orientation: A Meta-Analysis’, Frontiers in Human Neuroscience, vol. 12, 2018, Accessed: Oct. 20, 2022. [Online]. Available: https://www.frontiersin.org/articles/10.3389/fnhum.2018.00190
[36] M. M. Milczarek, S. D. Vann, and F. Sengpiel, ‘Spatial Memory Engram in the Mouse Retrosplenial Cortex’, Current Biology, vol. 28, no. 12, pp. 1975-1980.e6, Jun. 2018, doi: 10.1016/j.cub.2018.05.002.
[37] C. Trapanese, H. Meunier, and S. Masi, ‘What, where and when: spatial foraging decisions in primates’, Biological Reviews, vol. 94, no. 2, pp. 483–502, 2019, doi: 10.1111/brv.12462.
[38] A. G. Rosati, ‘Foraging Cognition: Reviving the Ecological Intelligence Hypothesis’, Trends in Cognitive Sciences, vol. 21, no. 9, pp. 691–702, Sep. 2017, doi: 10.1016/j.tics.2017.05.011.
[39] S. T. Parker, ‘Re-evaluating the extractive foraging hypothesis’, New Ideas in Psychology, vol. 37, pp. 1–12, Feb. 2015, doi: 10.1016/j.newideapsych.2014.11.001.
[40] T. Suddendorf and M. C. Corballis, ‘Mental time travel across the disciplines: The future looks bright’, Behavioral and Brain Sciences, vol. 30, no. 3, pp. 335–345, Jun. 2007, doi: 10.1017/S0140525X0700221X.
[41] L. Barrett, P. Henzi, and R. Dunbar, ‘Primate cognition: from “what now?” to “what if?”’, Trends in Cognitive Sciences, vol. 7, no. 11, Art. no. 11, Nov. 2003, doi: 10.1016/j.tics.2003.09.005.
[42] S.-L. Ding et al., ‘Comprehensive cellular-resolution atlas of the adult human brain’, J Comp Neurol, vol. 524, no. 16, pp. 3127–3481, Nov. 2016, doi: 10.1002/cne.24080.
[43] E. Armstrong, K. Zilles, G. Schlaug, and A. Schleicher, ‘Comparative aspects of the primate posterior cingulate cortex’, J. Comp. Neurol., vol. 253, no. 4, pp. 539–548, Nov. 1986, doi: 10.1002/cne.902530410.
[44] E. H. Willbrand et al., ‘Uncovering a tripartite landmark in posterior cingulate cortex’, Science Advances, vol. 8, no. 36, p. eabn9516, Sep. 2022, doi: 10.1126/sciadv.abn9516.
[45] M. F. Mason, M. I. Norton, J. D. Van Horn, D. M. Wegner, S. T. Grafton, and C. N. Macrae, ‘Wandering Minds: The Default Network and Stimulus-Independent Thought’, Science, vol. 315, no. 5810, pp. 393–395, Jan. 2007, doi: 10.1126/science.1131295.
[46] R. J. Maddock, A. S. Garrett, and M. H. Buonocore, ‘Remembering familiar people: the posterior cingulate cortex and autobiographical memory retrieval’, Neuroscience, vol. 104, no. 3, pp. 667–676, Jun. 2001, doi: 10.1016/S0306-4522(01)00108-7.
[47] R. J. Maddock, A. S. Garrett, and M. H. Buonocore, ‘Posterior cingulate cortex activation by emotional words: fMRI evidence from a valence decision task’, Hum Brain Mapp, vol. 18, no. 1, pp. 30–41, Jan. 2003, doi: 10.1002/hbm.10075.
[48] P. Qin and G. Northoff, ‘How is our self related to midline regions and the default-mode network?’, NeuroImage, vol. 57, no. 3, pp. 1221–1233, Aug. 2011, doi: 10.1016/j.neuroimage.2011.05.028.
[49] G. Northoff, A. Heinzel, M. de Greck, F. Bermpohl, H. Dobrowolny, and J. Panksepp, ‘Self-referential processing in our brain—A meta-analysis of imaging studies on the self’, NeuroImage, vol. 31, no. 1, pp. 440–457, May 2006, doi: 10.1016/j.neuroimage.2005.12.002.
[50] N. Hirono et al., ‘Hypofunction in the posterior cingulate gyrus correlates with disorientation for time and place in Alzheimer’s disease’, Journal of Neurology, Neurosurgery & Psychiatry, vol. 64, no. 4, pp. 552–554, Apr. 1998, doi: 10.1136/jnnp.64.4.552.
[51] J. M. Pearson, S. R. Heilbronner, D. L. Barack, B. Y. Hayden, and M. L. Platt, ‘Posterior cingulate cortex: adapting behavior to a changing world’, Trends in Cognitive Sciences, vol. 15, no. 4, pp. 143–151, Apr. 2011, doi: 10.1016/j.tics.2011.02.002.
[52] J. Brewer, K. Garrison, and S. Whitfield-Gabrieli, ‘What about the “Self” is Processed in the Posterior Cingulate Cortex?’, Frontiers in Human Neuroscience, vol. 7, 2013, Accessed: Oct. 17, 2022. [Online]. Available: https://www.frontiersin.org/articles/10.3389/fnhum.2013.00647
[53] A. Pfefferbaum et al., ‘Cerebral Blood Flow in Posterior Cortical Nodes of the Default Mode Network Decreases with Task Engagement but Remains Higher than in Most Brain Regions’, Cerebral Cortex, vol. 21, no. 1, pp. 233–244, Jan. 2011, doi: 10.1093/cercor/bhq090.
[54] O. Sporns, Networks of the brain. Cambridge, Mass.: MIT Press, 2011.
[55] R. L. Buckner, J. R. Andrews-Hanna, and D. L. Schacter, ‘The Brain’s Default Network’, Annals of the New York Academy of Sciences, vol. 1124, no. 1, pp. 1–38, 2008, doi: 10.1196/annals.1440.011.
[56] R. Leech, R. Braga, and D. J. Sharp, ‘Echoes of the Brain within the Posterior Cingulate Cortex’, J. Neurosci., vol. 32, no. 1, pp. 215–222, Jan. 2012, doi: 10.1523/JNEUROSCI.3689-11.2012.
[57] P. Hagmann et al., ‘Mapping the structural core of human cerebral cortex’, PLoS Biol, vol. 6, no. 7, p. e159, Jul. 2008, doi: 10.1371/journal.pbio.0060159.
[58] J. Smallwood et al., ‘Representing Representation: Integration between the Temporal Lobe and the Posterior Cingulate Influences the Content and Form of Spontaneous Thought’, PLOS ONE, vol. 11, no. 4, p. e0152272, Apr. 2016, doi: 10.1371/journal.pone.0152272.
[59] U. Hasson, J. Chen, and C. J. Honey, ‘Hierarchical process memory: memory as an integral component of information processing’, Trends in Cognitive Sciences, vol. 19, no. 6, pp. 304–313, Jun. 2015, doi: 10.1016/j.tics.2015.04.006.
[60] E. Valenstein, D. Bowers, M. Verfaellie, K. M. Heilman, A. Day, and R. T. Watson, ‘Retrosplenial amnesia’, Brain, vol. 110 ( Pt 6), pp. 1631–1646, Dec. 1987, doi: 10.1093/brain/110.6.1631.
[61] P. L. St Jacques, M. A. Conway, M. W. Lowder, and R. Cabeza, ‘Watching my mind unfold versus yours: an fMRI study using a novel camera technology to examine neural differences in self-projection of self versus other perspectives’, J Cogn Neurosci, vol. 23, no. 6, pp. 1275–1284, Jun. 2011, doi: 10.1162/jocn.2010.21518.
[62] K. Friston, ‘Am I Self-Conscious? (Or Does Self-Organization Entail Self-Consciousness?)’, Frontiers in Psychology, vol. 9, p. 579, Apr. 2018, doi: 10.3389/fpsyg.2018.00579.
[63] G. Pezzulo, T. Parr, and K. Friston, ‘The evolution of brain architectures for predictive coding and active inference’, Philosophical Transactions of the Royal Society B: Biological Sciences, vol. 377, no. 1844, p. 20200531, Feb. 2022, doi: 10.1098/rstb.2020.0531.
[64] A. D’Argembeau et al., ‘Distinct Regions of the Medial Prefrontal Cortex Are Associated with Self-referential Processing and Perspective Taking’, Journal of Cognitive Neuroscience, vol. 19, no. 6, pp. 935–944, Jun. 2007, doi: 10.1162/jocn.2007.19.6.935.
[65] J. Kurczek et al., ‘Differential contributions of hippocampus and medial prefrontal cortex to self-projection and self-referential processing’, Neuropsychologia, vol. 73, pp. 116–126, Jul. 2015, doi: 10.1016/j.neuropsychologia.2015.05.002.
[66] M. van Buuren, T. E. Gladwin, B. B. Zandbelt, R. S. Kahn, and M. Vink, ‘Reduced functional coupling in the default-mode network during self-referential processing’, Human Brain Mapping, vol. 31, no. 8, pp. 1117–1127, 2010, doi: 10.1002/hbm.20920.
[67] Tulving, Endel, ‘Episodic Memory: From Mind to Brain | Annual Review of Psychology’, 2002. https://www.annualreviews.org/doi/10.1146/annurev.psych.53.100901.135114 (accessed Oct. 23, 2022).
[68] R. L. Buckner and D. C. Carroll, ‘Self-projection and the brain’, Trends in Cognitive Sciences, vol. 11, no. 2, pp. 49–57, Feb. 2007, doi: 10.1016/j.tics.2006.11.004.
[69] K. A. Bard, B. K. Todd, C. Bernier, J. Love, and D. A. Leavens, ‘Self-Awareness in Human and Chimpanzee Infants: What Is Measured and What Is Meant by the Mark and Mirror Test?’, Infancy, vol. 9, no. 2, Art. no. 2, 2006, doi: 10.1207/s15327078in0902_6.
[70] D. L. Butler and J. R. Anderson, ‘Visual Self-Recognition’, in Encyclopedia of Animal Cognition and Behavior, J. Vonk and T. Shackelford, Eds. Cham: Springer International Publishing, 2018, pp. 1–9. doi: 10.1007/978-3-319-47829-6_742-1.
[71] D. J. Povinelli, ‘The Self: Elevated in Consciousness and Extended in Time’, in The Self in Time, Psychology Press, 2001.
[72] T. Suddendorf, J. Redshaw, and A. Bulley, The Invention of Tomorrow: A Natural History of Foresight. New York: Basic Books, 2022.
[73] S. Hirata, K. Fuwa, and M. Myowa, ‘Chimpanzees recognize their own delayed self-image’, Royal Society open science, vol. 4, no. 8, Art. no. 8, 2017, doi: https://doi.org/10.1098/rsos.170370.
[74] I. R. Kolvoort, S. Wainio-Theberge, A. Wolff, and G. Northoff, ‘Temporal integration as “common currency” of brain and self-scale-free activity in resting-state EEG correlates with temporal delay effects on self-relatedness’, Human Brain Mapping, vol. 41, no. 15, pp. 4355–4374, 2020, doi: 10.1002/hbm.25129.
[75] G. Northoff, ‘Personal Identity and Cortical Midline Structure (CMS): Do Temporal Features of CMS Neural Activity Transform Into “Self-Continuity”?’, Psychological Inquiry, vol. 28, no. 2–3, pp. 122–131, Jul. 2017, doi: 10.1080/1047840X.2017.1337396.
[76] A. Wolff et al., ‘The temporal signature of self: Temporal measures of resting-state EEG predict self-consciousness’, Human Brain Mapping, vol. 40, no. 3, pp. 789–803, 2019, doi: 10.1002/hbm.24412.
[77] G. Northoff and D. Gorini, ‘The hierarchical semantics of self’, in The Routledge Handbook of Semiosis and the Brain, 1st ed., New York: Routledge, 2022, pp. 387–405. doi: 10.4324/9781003051817-30.
[78] G. Northoff and A. Scalabrini, ‘“Project for a Spatiotemporal Neuroscience” – Brain and Psyche Share Their Topography and Dynamic’, Frontiers in Psychology, vol. 12, 2021, Accessed: May 20, 2022. [Online]. Available: https://www.frontiersin.org/article/10.3389/fpsyg.2021.717402
[79] P. Qin, M. Wang, and G. Northoff, ‘Linking bodily, environmental and mental states in the self—A three-level model based on a meta-analysis’, Neuroscience & Biobehavioral Reviews, vol. 115, pp. 77–95, Aug. 2020, doi: 10.1016/j.neubiorev.2020.05.004.
[80] J. Sui and G. W. Humphreys, ‘The Integrative Self: How Self-Reference Integrates Perception and Memory’, Trends in Cognitive Sciences, vol. 19, no. 12, pp. 719–728, Dec. 2015, doi: 10.1016/j.tics.2015.08.015.
[81] J. Sui, X. He, and G. W. Humphreys, ‘Perceptual effects of social salience: Evidence from self-prioritization effects on perceptual matching’, Journal of Experimental Psychology: Human Perception and Performance, vol. 38, pp. 1105–1117, 2012, doi: 10.1037/a0029792.
[82] R. Saxe and L. J. Powell, ‘It’s the Thought That Counts: Specific Brain Regions for One Component of Theory of Mind’, Psychol Sci, vol. 17, no. 8, pp. 692–699, Aug. 2006, doi: 10.1111/j.1467-9280.2006.01768.x.
[83] A. S. Alexander et al., ‘Adaptive integration of self-motion and goals in posterior parietal cortex’, Cell Reports, vol. 38, no. 10, p. 110504, Mar. 2022, doi: 10.1016/j.celrep.2022.110504.
[84] A. Newen, ‘The Embodied Self, the Pattern Theory of Self, and the Predictive Mind’, Frontiers in Psychology, vol. 9, 2018, Accessed: Oct. 25, 2022. [Online]. Available: https://www.frontiersin.org/articles/10.3389/fpsyg.2018.02270
[85] A. K. Seth, ‘Interoceptive inference, emotion, and the embodied self’, Trends in Cognitive Sciences, vol. 17, no. 11, pp. 565–573, Nov. 2013, doi: 10.1016/j.tics.2013.09.007.
[86] M. P. van den Heuvel, E. T. Bullmore, and O. Sporns, ‘Comparative Connectomics’, Trends in Cognitive Sciences, vol. 20, no. 5, pp. 345–361, May 2016, doi: 10.1016/j.tics.2016.03.001.
[87] E. Barsotti, A. Correia, and A. Cardona, ‘Neural architectures in the light of comparative connectomics’, Current Opinion in Neurobiology, vol. 71, pp. 139–149, Dec. 2021, doi: 10.1016/j.conb.2021.10.006.
[88] L. E. Suárez et al., ‘A connectomics-based taxonomy of mammals’. bioRxiv, p. 2022.03.11.483995, Mar. 12, 2022. doi: 10.1101/2022.03.11.483995.
[89] C. Yokoyama et al., ‘Comparative connectomics of the primate social brain’, NEUROIMAGE, vol. 245. ACADEMIC PRESS INC ELSEVIER SCIENCE, 525 B ST, STE 1900, SAN DIEGO, CA 92101-4495 USA, Dec. 15, 2021. doi: 10.1016/j.neuroimage.2021.118693.
[90] A. Reiner et al., ‘Revised nomenclature for avian telencephalon and some related brainstem nuclei’, Journal of Comparative Neurology, vol. 473, no. 3, pp. 377–414, 2004, doi: 10.1002/cne.20118.
[91] E. D. Jarvis et al., ‘Avian brains and a new understanding of vertebrate brain evolution’, Nat Rev Neurosci, vol. 6, no. 2, Art. no. 2, Feb. 2005, doi: 10.1038/nrn1606.
[92] O. Güntürkün and T. Bugnyar, ‘Cognition without Cortex’, Trends in Cognitive Sciences, vol. 20, no. 4, pp. 291–303, Apr. 2016, doi: 10.1016/j.tics.2016.02.001.
[93] O. Güntürkün, ‘The avian “prefrontal cortex” and cognition’, Current Opinion in Neurobiology, vol. 15, no. 6, pp. 686–693, Dec. 2005, doi: 10.1016/j.conb.2005.10.003.
[94] O. Güntürkün, K. von Eugen, J. Packheiser, and R. Pusch, ‘Avian pallial circuits and cognition: A comparison to mammals’, Current Opinion in Neurobiology, vol. 71, pp. 29–36, Dec. 2021, doi: 10.1016/j.conb.2021.08.007.
[95] N. S. Clayton and N. J. Emery, ‘Avian Models for Human Cognitive Neuroscience: A Proposal’, Neuron, vol. 86, no. 6, pp. 1330–1342, Jun. 2015, doi: 10.1016/j.neuron.2015.04.024.
[96] M. Stacho et al., ‘A cortex-like canonical circuit in the avian forebrain’, Science, vol. 369, no. 6511, p. eabc5534, Sep. 2020, doi: 10.1126/science.abc5534.
[97] N. J. Emery and N. S. Clayton, ‘The Mentality of Crows: Convergent Evolution of Intelligence in Corvids and Apes’, Science, vol. 306, no. 5703, pp. 1903–1907, Dec. 2004, doi: 10.1126/science.1098410.
[98] L. Baciadonna, F. M. Cornero, N. J. Emery, and N. S. Clayton, ‘Convergent evolution of complex cognition: Insights from the field of avian cognition into the study of self-awareness’, Learn Behav, vol. 49, no. 1, pp. 9–22, Mar. 2021, doi: 10.3758/s13420-020-00434-5.
[99] N. J. Emery and N. S. Clayton, ‘Comparative Social Cognition’, Annu Rev Psychol, vol. 60, pp. 87–113, 2009.
[100] N. S. Clayton and A. Dickinson, ‘Episodic-like memory during cache recovery by scrub jays’, Nature, vol. 395, no. 6699, Art. no. 6699, Sep. 1998, doi: 10.1038/26216.
[101] N. J. Emery, J. M. Dally, and N. S. Clayton, ‘Western scrub-jays (Aphelocoma californica) use cognitive strategies to protect their caches from thieving conspecifics’, Anim Cogn, vol. 7, no. 1, pp. 37–43, Jan. 2004, doi: 10.1007/s10071-003-0178-7.
[102] ‘Effects of experience and social context on prospective caching strategies by scrub jays’, Nature, Nov. 2001, doi: 10.1038/news011122-13.
[103] H. Prior, A. Schwarz, and O. Güntürkün, ‘Mirror-Induced Behavior in the Magpie (Pica pica): Evidence of Self-Recognition’, PLOS Biology, vol. 6, no. 8, p. e202, Aug. 2008, doi: 10.1371/journal.pbio.0060202.
[104] K. F. Brecht, J. Müller, and A. Nieder, ‘Carrion crows (Corvus corone corone) fail the mirror mark test yet again.’, Journal of Comparative Psychology, vol. 134, no. 4, pp. 372–378, Nov. 2020, doi: 10.1037/com0000231.
[105] L. Baciadonna, G. M. Jerwood, B. G. Farrar, N. S. Clayton, and N. J. Emery, ‘Investigation of mirror-self recognition in ravens (Corvus corax)’, Journal of Comparative Psychology, vol. 136, pp. 194–198, 2022, doi: 10.1037/com0000319.
[106] T. Freeberg, ‘On mark-test replication and mirror self-recognition in magpies.’, Journal of Comparative Psychology, vol. 134, pp. 361–362, Nov. 2020, doi: 10.1037/com0000256.
[107] M. Soler, J. M. Colmenero, T. Pérez-Contreras, and J. M. Peralta-Sánchez, ‘Replication of the mirror mark test experiment in the magpie (Pica pica) does not provide evidence of self-recognition.’, Journal of Comparative Psychology, vol. 134, no. 4, pp. 363–371, Nov. 2020, doi: 10.1037/com0000223.
[108] K. F. Brecht and A. Nieder, ‘Parting self from others: Individual and self-recognition in birds’, Neuroscience & Biobehavioral Reviews, vol. 116, pp. 99–108, Sep. 2020, doi: 10.1016/j.neubiorev.2020.06.012.
[109] M. Soler, T. Pérez-Contreras, and J. M. Peralta-Sánchez, ‘Mirror-Mark Tests Performed on Jackdaws Reveal Potential Methodological Problems in the Use of Stickers in Avian Mark-Test Studies’, PLOS ONE, vol. 9, no. 1, p. e86193, Jan. 2014, doi: 10.1371/journal.pone.0086193.
[110] Y. Atoji and J. M. Wild, ‘Afferent and efferent connections of the dorsolateral corticoid area and a comparison with connections of the temporo-parieto-occipital area in the pigeon (Columba livia)’, Journal of Comparative Neurology, vol. 485, no. 2, pp. 165–182, 2005, doi: 10.1002/cne.20490.
[111] M. Shanahan, The network architecture of the avian brain and its role in cognition. 2012.
[112] R. O. Prum et al., ‘A comprehensive phylogeny of birds (Aves) using targeted next-generation DNA sequencing’, Nature, vol. 526, no. 7574, Art. no. 7574, Oct. 2015, doi: 10.1038/nature15697.
[113] H. Lu, Q. Zou, H. Gu, M. E. Raichle, E. A. Stein, and Y. Yang, ‘Rat brains also have a default mode network’, Proceedings of the National Academy of Sciences, vol. 109, no. 10, pp. 3979–3984, Mar. 2012, doi: 10.1073/pnas.1200506109.
[114] D. Popa, A. T. Popescu, and D. Paré, ‘Contrasting activity profile of two distributed cortical networks as a function of attentional demands’, J Neurosci, vol. 29, no. 4, pp. 1191–1201, Jan. 2009, doi: 10.1523/jneurosci.4867-08.2009.
[115] J. M. Stafford et al., ‘Large-scale topology and the default mode network in the mouse connectome’, Proc Natl Acad Sci U S A, vol. 111, no. 52, pp. 18745–18750, Dec. 2014, doi: 10.1073/pnas.1404346111.
[116] J. L. Vincent et al., ‘Intrinsic functional architecture in the anaesthetized monkey brain’, Nature, vol. 447, no. 7140, Art. no. 7140, May 2007, doi: 10.1038/nature05758.
[117] F. Guo et al., ‘Frequency-dependent alterations in the default mode network during general anesthesia: a study in the rat’, In Review, preprint, Jun. 2022. doi: 10.21203/rs.3.rs-1729390/v1.
[118] X. Zhang, ‘Effects of Anesthesia on Cerebral Blood Flow and Functional Connectivity of Nonhuman Primates’, Veterinary Sciences, vol. 9, no. 10, Art. no. 10, Oct. 2022, doi: 10.3390/vetsci9100516.
[119] T. Moher Alsady, E. M. Blessing, and F. Beissner, ‘MICA—A toolbox for masked independent component analysis of fMRI data’, Human Brain Mapping, vol. 37, no. 10, pp. 3544–3556, 2016, doi: 10.1002/hbm.23258.
[120] S. B. Eickhoff, B. T. T. Yeo, and S. Genon, ‘Imaging-based parcellations of the human brain’, Nat Rev Neurosci, vol. 19, no. 11, Art. no. 11, Nov. 2018, doi: 10.1038/s41583-018-0071-7.
[121] E. Bullmore and O. Sporns, ‘Complex brain networks: graph theoretical analysis of structural and functional systems’, Nat Rev Neurosci, vol. 10, no. 3, Art. no. 3, Mar. 2009, doi: 10.1038/nrn2575.
[122] M. Rubinov and O. Sporns, ‘Complex network measures of brain connectivity: Uses and interpretations’, NeuroImage, vol. 52, no. 3, pp. 1059–1069, Sep. 2010, doi: 10.1016/j.neuroimage.2009.10.003.
[123] M. Kaiser, ‘Changing Connectomes’, p. 271.
[124] M. Kaiser, ‘A tutorial in connectome analysis: Topological and spatial features of brain networks’, NeuroImage, vol. 57, no. 3, pp. 892–907, Aug. 2011, doi: 10.1016/j.neuroimage.2011.05.025.
[125] C. Higgins et al., ‘Replay bursts in humans coincide with activation of the default mode and parietal alpha networks’, Neuron, vol. 109, no. 5, pp. 882-893.e7, Mar. 2021, doi: 10.1016/j.neuron.2020.12.007.
[126] J. L. McClelland and R. C. O’Reilly, ‘Why There Are Complementary Learning Systems in the Hippocampus and Neocortex:InsightsFrom the Successesand Failuresof Connectionist Models of Learning and Memory’, p. 39.
[127] Z. Kurth-Nelson et al., ‘Replay and compositional computation’. arXiv, Sep. 15, 2022. doi: 10.48550/arXiv.2209.07453.
[128] D. S. Margulies et al., ‘Situating the default-mode network along a principal gradient of macroscale cortical organization’, Proceedings of the National Academy of Sciences, vol. 113, no. 44, pp. 12574–12579, Nov. 2016, doi: 10.1073/pnas.1608282113.
[129] K. Kaefer, F. Stella, B. L. McNaughton, and F. P. Battaglia, ‘Replay, the default mode network and the cascaded memory systems model’, Nat Rev Neurosci, vol. 23, no. 10, Art. no. 10, Oct. 2022, doi: 10.1038/s41583-022-00620-6.
Comments