Brain-body size divergence in birds: An evolutionary, morphological and ecological case
- Akseli Ilmanen
- Jan 16, 2023
- 13 min read
Updated: Jul 10, 2023
For my evolution class, I had to write an essay on the question “Is Cope’s Rule- the tendency of lineages to evolve large size over time- real- and if so, what drives it?” As I am currently (a bit) obsessed with bird brain evolution (particularly Corvids), the essay went in that direction.
Named after Edward Cope, Cope’s rule states that species in mammalian clades tend to increase in size over the Cenozoic era (Hone and Benton, 2005). The idea has also been applied to other periods and groups (Kingsolver and Pfennig, 2004). Two popular critiques of Cope’s rule frame it as a passive diversification process (McShea, 1994) or a statistical artefact caused by founder populations (Stanley, 1973). In the following, I will engage with the critiques above and the more particular argument that morphological specialization in large animals may limit their evolvability (ibid). This will bridge to some evolutionary-developmental biology concepts. From there, I will discuss what drives body size[1] increases in extant[2] flying birds (Neoaves) and extinct pterosaurs. Next, I will connect morphology to ecology through the ‘early burst’ model and ‘morphospaces’. By then we should have a very rich conceptual understanding of body size constraints, which we will explore practically by the example of bird brain evolution.
Is Cope’s rule a passive trend of diversification (Fig 1A.) or an active trend of directional selection.[3] (Fig 1B.)? For now, consider the graph in Fig 1C. as the base case. This could be the diversification of a clade over time where at time point zero all organisms have the same size body size. Examples may be adaptive radiation[4] of surviving clades after a mass extinction event or founder populations on previously uninhabited islands (Bergstrom and Dugatkin, 2016).
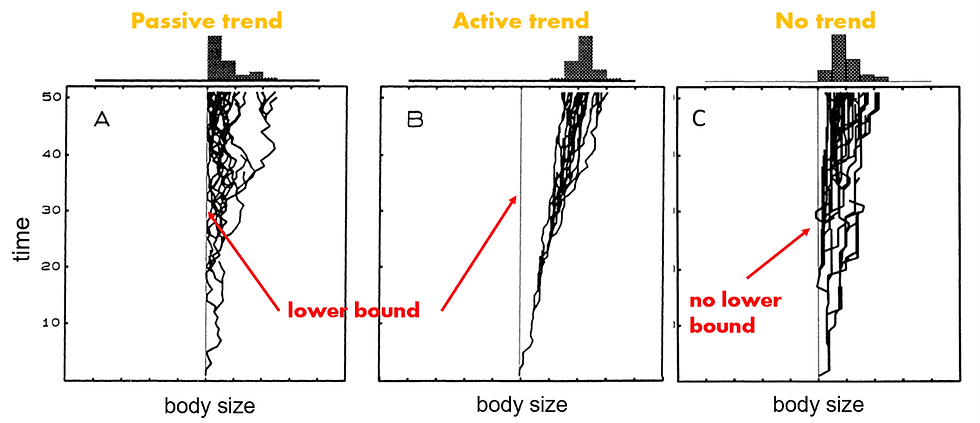
Now, unlike in Fig 1C, 1A. and 1B. have a lower bound for body size. For example, birds weigh at least 4–5 grams[5] to support the minimum space required for some fundamental organ systems (Stanley, 1973) The ‘passive trend’ argument (Fig 1A.) is that as the clade diversifies over time when there is a lower bound on how small individuals in that clade can be, it seems as if over time the lineages evolve to larger sizes (ibid). However, if we contrast this with the ‘active trend’ or directional selection, we may notice that both the lower bound and upper bound increase in size. In other words, the trait values for size within each subclade shifted “in parallel” (Bergstrom and Dugatkin, 2016, p.562).
There is disagreement as to how universal the active trend is. Some emphasize the adaptive benefits of large size in prey-predator relations, competition for food and mates, or higher intelligence due to larger brain size (Hone and Benton, 2005). These advantages can be selected for either by shifting the body size distributions of existing species in a clade to the right or higher rates of speciation in large and extinction in smaller animals lead to a “variational process” favouring larger sizes over time (Bergstrom and Dugatkin, 2016, p.562). On the opposite side, Stanley (1973) points out that exactly because of this overemphasis on fitness and ecological specialization, Edward Cope and others failed to acknowledge the role of morphological specialization in the passive trend. He argues that larger animals have more specialized morphologies (ibid). Consider for example the functional role of an elephant’s trunk or spider monkeys’ (Ateles) brachiating forelimbs and prehensile tails as structural specializations for clinging and locomotion. It appears unlikely that they could give rise to descendent species without these characteristic morphological feature.[6]
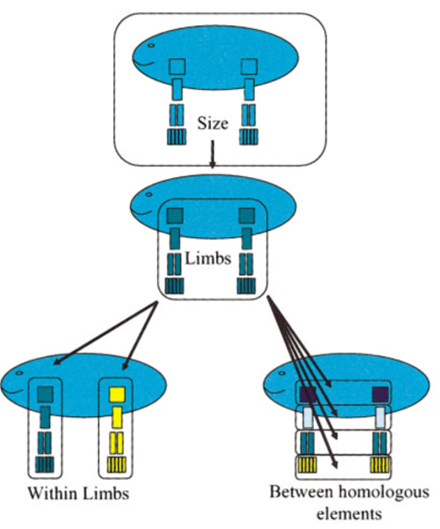
Stanley’s (1973) morphological specialization argument is both supported and contradicted by the evo-devo literature. Pro Stanley (1973), larger animals tend to employ a K-selection strategy; they produce fewer offspring with high parental investment (Hone and Benton, 2005). Combined with longer lifespans, this gives far fewer chances for speciation to larger sizes to occur. Furthermore, larger bodies, necessitated by their scale, might include more ‘modules’ (Fig 2.), that need to carry out ‘morphological integration’ processes (Olson and Miller, 1999) such as coordinating the growth of both fore- and hindlimbs (Hallgrímsson, Willmore and Hall, 2002). Hence, during canalization processes[7], large organisms might show “high degrees of phenotypic stability” (ibid., p.142), as their hierarchically modular and integrated morphology requires a very particular developmental pathway. In Fig 3., this could be visually represented by steep slopes and few neighbouring valleys, suggesting the rarity or lack of alternative developmental pathways.

Contra Stanley (1973), modularity may increase evolvability by decreasing pleiotropic effects[8] across modules, and thus “among traits that are not functionally related” (Hallgrímsson, Willmore, and Hall, 2002, pp.133). So in most cases, where pleiotropy is disadvantageous, this limits their effects to some modules. And in cases, where pleiotropic genes would increase fitness in some modules but be highly deleterious in others modules, their dissociation increases evolvability. Also, contra Stanley (1973), using a computational evo-devo approach Kriegman, Cheney, and Bongard (2018) argue for ‘differential canalization’. They simulate a virtual environment, where robots can evolve their morphology to maximize movement speed (see video). In the most successful simulations, although the morphology of the robots becomes genetically assimilated[9] over time, this is not the case for their ’developmental controllers’ (ibid). Returning to Stanley’s (1973) argument, this may be understood as: Although body size or other morphological specialisation are genetically assimilated, the underlying developmental mechanisms controlling them may be separate, plastic, and could in theory facilitate morphological change. Moving forward, we have a contested characterization of Cope’s rule and should pay attention to ecology, morphology, and evo-devo.
Let’s discuss birds. Using Fig 1., we highlighted the role of boundary effects for small body size. But do birds even start close to the boundary, at a small size? During the origin of modern birds, at the Cretaceous–Palaeogene (K–Pg) extinction, there is an argument, known as the lilliput effect, that mostly small birds survived and subsequently diversified (Berv and Field, 2018). This contrasts with Stanley’s (1973, p.3) rhetorical point making us consider Cope’s rule in a scenario where the initial ancestor was “the size of a horse”. His point has some relevance, as the Archaeopteryx (Fig 4), a ‘transitional fossil’ (Lyson et al., 2010) between non-avian therapods and modern birds[10], probably weighed around 220–330g (Yalden, 1984), which is comparable with the relatively large Eurasian magpies.[11] So, this goes against the Cope-ian story of evolution from small size. Is there any evidence for evolution towards large size in birds or pterosaurs?
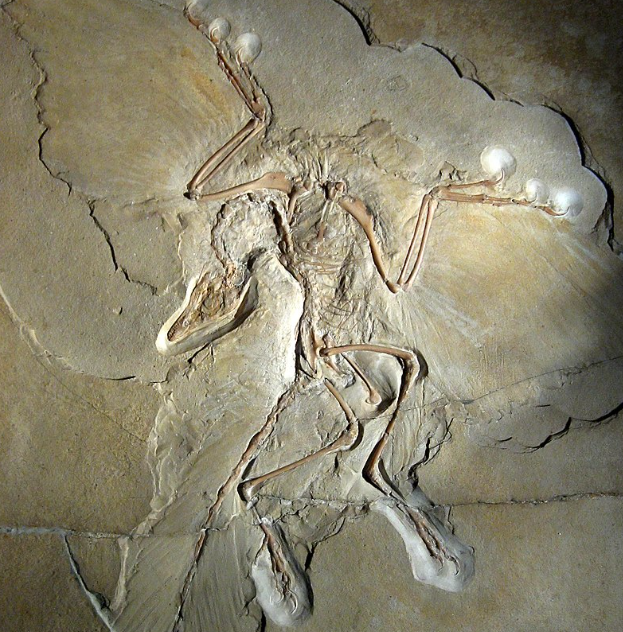
Sampling from fore- and hindlimb bones of Mesozoic birds, Hone et al. (2008) argued that within two Mesozoic bird clades body size increased over a period of 70 million years. However, when Butler and Goswami (2008) used phylogenetic reconstruction techniques to assess ancestor–descendant relationships (Alroy, 2000) with the data of Hone et al. (2008), they were unable to replicate the findings. Similarly, Benson et al. (2014) claim that in the last 65–85 million years before their extinction in the K–Pg boundary, pterosaurs’ body size increased from <1.6 metres body size to wingspans of 3–10 metres. They argued that, as this was during the K–Pg boundary, temporally coincident with the ‘passive’ body size diversification of pygostylian birds, this led to bird-pterosaurs competition, where birds “may have excluded pterosaurs from the ecological niches available to smaller flying animals, causing selective extinction of smaller pterosaurs, and also possibly causing active selection for larger body size in pterosaur evolution” (Benson et al., 2014, p.3). One limitation here is that fossil records are biased towards larger organisms, as these are more well-preserved and easily discovered (Bergstrom and Dugatkin, 2016).
Moving away from forces for larger bodies, let’s consider some constraints to body size. More specifically, we will ask why there is a right-skewed distribution of body mass in modern birds (Bokma, 2002) — see Fig 5. If we buy into ‘active’ Cope’s law, then we should expect a left-skewed distribution or at least a distribution that is shifting leftwards over time. (To imagine this visually, compare Fig 1C with 1B, and consider how the distribution is shifting leftwards). So, how do we explain the right-skewed distribution (Fig 5.)? Is this a passive trend or might there be an ‘active constraint’ on body size? The earlier point about a K-selection strategy points toward this. And the ability for flight induces some limits on the right side of the distribution (Fig 5). To fly as a heavier bird requires the allometric scaling of wings, and as Gould (1966) argues there may be an upper limit of around 15kg.
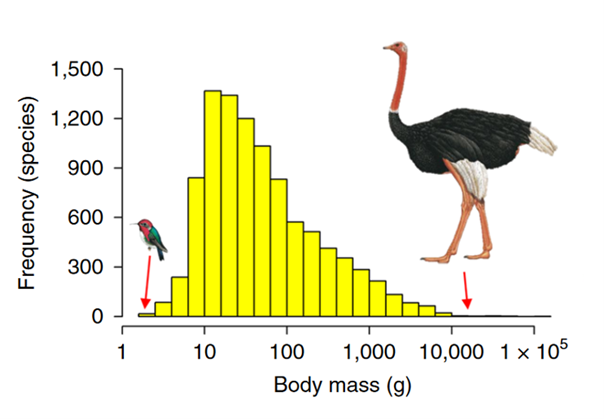
But arguably the most important factor constraining body size might be ecology. To better understand this point, we have to understand how ecology is a constraint over time. In theories about adaptative radiation4, there is sometimes an implicit assumption that diversification is always accompanied by higher morphological diversity (Wills, Briggs and Fortey, 1994). In an ‘early burst’ model (EBM)[12] (Gavrilets and Losos, 2009), all the morphological innovations happen very quickly filling all the ‘empty space’ of ecological niches (Fig 6. left).
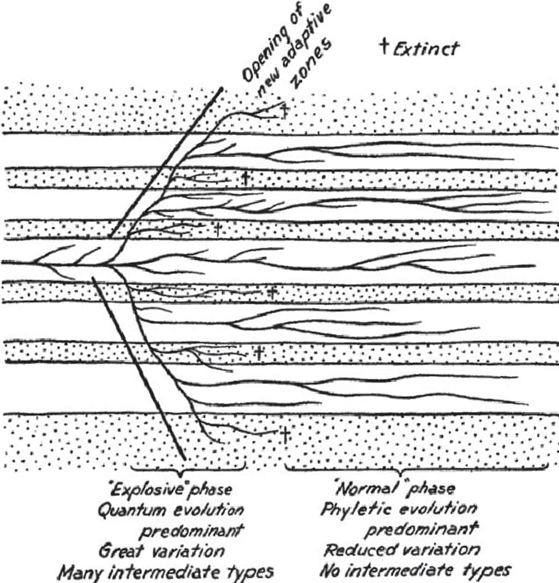
This is followed by a period of ‘niche conservatism’ (Fig 6. right), made robust by canalization (Fig 3.), where all the viable ‘morphospaces’ (Fig 7.) have been found. Thus, there is little space for drastic morphological innovations within sub-clades. Returning to Fig 5., we could argue the right-skewed distribution of body mass simply reflects a right-skewed distribution of available ecological niches. They are matched by a ‘form-function’ relationship (Pigot et al., 2020), where all the ‘small forms’ fit into more niches than the ‘large forms’. But this argument of matching ecological with morphological distributions only works if all the ‘empty ecological niche space’ have already been filled. This relies on the EBM.

The EBM has only partial empirical support. Navalón et al. (2022) compared bird morphological data. with Brownian motion models. They found fewer group-specific disparities in the former than latter (Fig 8). Contra EBM, this suggests the morphospace is not just quickly filling in the ‘empty space’ (similar to how gas particles distribute in a room) but might remain quite centered (similar to the yellow cluster in Fig 7. left). Pro EBM, when comparing Neoave clades, Navalón et al. (2022) found an early morphological divergence between waterbirds (Aequornithes) and landbirds (Telluraves). Contra EBM and Stanley (1973), waterbirds’ morphospaces changed considerably long after the K–Pg boundary, including large body size and coarse-grained locomotory adaptations (Navalón et al., 2022). For landbirds’, and their suborder Passeriformes[13], the case is quite complicated. Pro EBM, they showed only low-scaled morphological changes (ibid.) but molecular phylogenetic methods (Jetz et al., 2012) showed high long-term diversification rate, particularly in the last 50 million years. Navalón et al. (2022) argue that this could be driven by geographical differentiation and behavioural divergences with only subtle ecomorphological changes. This is very interesting with respect to bird brain evolution.
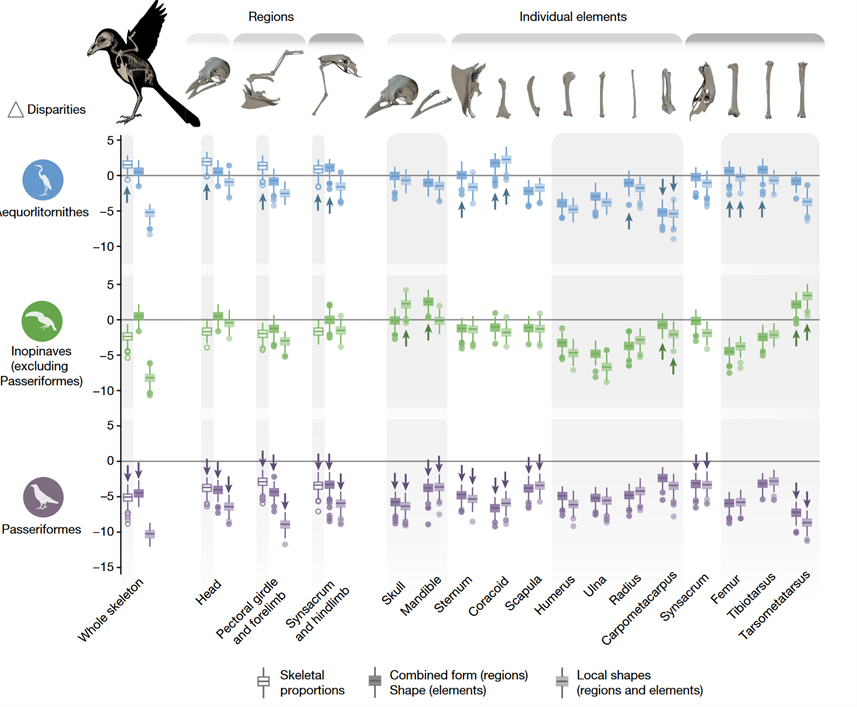
Onto bird brain evolution. For simplicity, we will restrict ourselves to passerine birds (Passeriformes order) only. To recap, in line with EBM (Fig 6.), passerines found their ecological niche and morphospaces early. Since then, maybe also due to canalization (Fig 3.), their coarse-grained morphologies (i.e., body size) have changed comparatively little. This is despite them long-term having very high diversification rates, potentially reflecting geographical differentiation and/or behavioural speciation.
Now what is interesting is that the ratio of brain to body size (encephalization quotients) has actually been increasing quite a lot over therapod-bird evolution (Fig 9.), including for passerines (particularly corvids). Ksepka et al. (2020) explain this mainly in terms of diversification towards equally sized bodies with larger brain size, or constant brain sizer but smaller bodies. So, although body size and other coarse-grained morphologies did not change, brain size did. This is evidence of non-allometric brain–body scaling. Indeed, there is research suggesting that in birds (and mammals) there are weaker developmental constraints on brain-body allometry (Tsuboi et al., 2018). Although this is very speculative, this might be through mechanisms such as ‘differential canalization’ (discussed earlier) or evolutionary selection on ‘modules’ (Fig 2.) responsible for brain-body morphological integration. Moreover, the fact that avian neurons consume three times less glucose than mammalian neurons (von Eugen et al., 2022) might reflect selection pressures for bird brains with high neuronal densities (Olkowicz et al., 2016) but limits on brain volume[14].
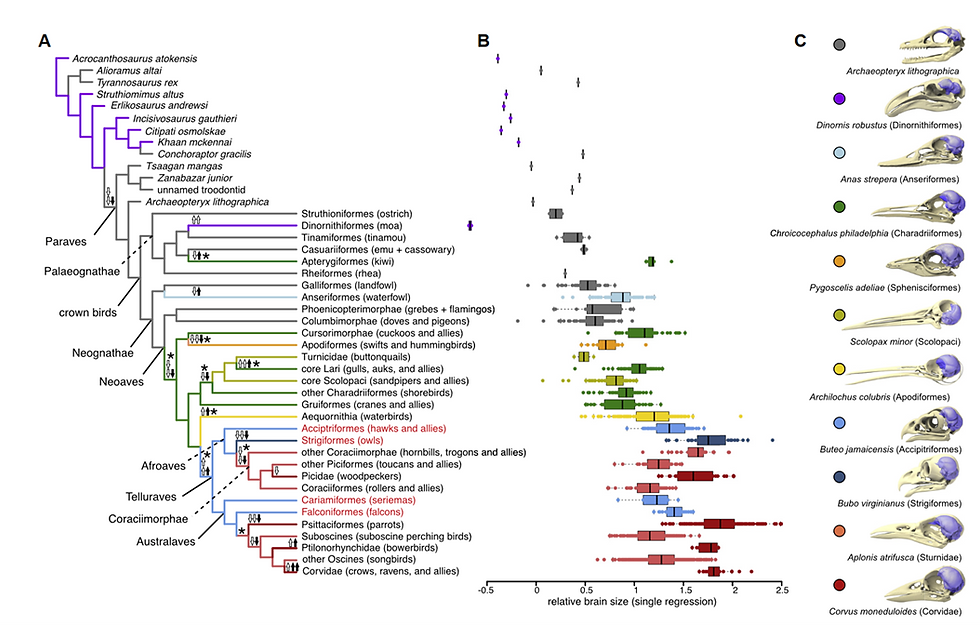
In conclusion, the case for ‘active’ Cope’s rule in Neoaves and pterosaurs has only very limited archaeological support. Indeed, on the opposite, there is a good case for an ‘active constraint’ on body size after adaptive radiation in the K–Pg boundary. Potentially, due to this constraint, bird evolution has actively selected for brain-body divergence and neuronally dense architectures.
Footnotes
[1]In the case of birds, body size could refer to their total length, body mass, wingspan, etc. (Hone and Benton, 2005). With ‘body size’ or ‘size’ I mean a broad composite of these.
[2] Extant means still in existence (not extinct).
[3] Directional selection is characterized by a shift in allele frequencies over time favouring one extreme phenotype over other phenotypes (Hamilton, 2009).
[4] “Adaptive radiation has two components: the production of new species (speciation) and the adaptation of constituent species to a diversity of ecological niches”. (Gavrilets and Losos, 2009, p.732)
[5] There are some exceptions in hummingbirds and others, who can have slightly lower weights, by having very high metabolic efficiency rates (Stanley, 1973).
[6] One could say they lack ‘morphological evolvability’ (Cooney and Thomas, 2021) or ‘versatility’ (Liem and Osse, 1975).
[7] Canalization is the extent of robustness by which a population produces the same phenotype despite environmental or genotypic variability (Scharloo, 1991). See also Fig 3.
[8] Pleiotropy is when one locus in the genome affects more than one trait (Hamilton, 2009).
[9] Genetic assimilation is the process where a genetic change initially caused by environmental factors becomes incorporated into a population’s genetic makeup (Waddington, 1953).
[10] Modern birds did not evolve from the (flying) pterosaurs but feathered, non-avian Deinonychosauria (Langston, 1981).
[11] Arguably after the recent discovery of several small, feathered therapods in China, it has been debated whether the Archaeopteryx is the ancestor of modern birds or their relative (Xu et al., 2011).
[12] We could also call this the early-niche filling model.
[13] Although Passeriformes are only of many clades of landbirds (Telluraves), they make up 50% of all extant bird species.
[14] Clearly, brain volume or neuronal density is not everything. More important to intelligence might be qualitative aspects of the brain such as connectivity or various geometric morphometrics (Marugán-Lobón, Watanabe and Kawabe, 2016)
References
Alroy, J., 2000. Understanding the Dynamics of Trends within Evolving Lineages. Paleobiology, 26(3), pp.319–329.
Benson, R.B.J., Frigot, R.A., Goswami, A., Andres, B. and Butler, R.J., 2014. Competition and constraint drove Cope’s rule in the evolution of giant flying reptiles. Nature Communications [Online], 5(1), p.3567. Available from: https://doi.org/10.1038/ncomms4567.
Bergstrom, C.T. and Dugatkin, L.A., 2016. Evolution. Second edition. New York: W. W. Norton & Company.
Berv, J.S. and Field, D.J., 2018. Genomic Signature of an Avian Lilliput Effect across the K-Pg Extinction. Systematic Biology [Online], 67(1), pp.1–13. Available from: https://doi.org/10.1093/sysbio/syx064.
Bokma, F., 2002. A Statistical Test of Unbiased Evolution of Body Size in Birds. Evolution [Online], 56(12), pp.2499–2504. Available from: https://doi.org/10.1111/j.0014-3820.2002.tb00174.x.
Butler, R.J. and Goswami, A., 2008. Body size evolution in Mesozoic birds: little evidence for Cope’s rule. Journal of Evolutionary Biology [Online], 21(6), pp.1673–1682. Available from: https://doi.org/10.1111/j.1420-9101.2008.01594.x.
Cooney, C.R., Bright, J.A., Capp, E.J.R., Chira, A.M., Hughes, E.C., Moody, C.J.A., Nouri, L.O., Varley, Z.K. and Thomas, G.H., 2017. Mega-evolutionary dynamics of the adaptive radiation of birds. Nature [Online], 542(7641), pp.344–347. Available from: https://doi.org/10.1038/nature21074.
Cooney, C.R. and Thomas, G.H., 2021. Heterogeneous relationships between rates of speciation and body size evolution across vertebrate clades. Nature Ecology & Evolution [Online], 5(1), pp.101–110. Available from: https://doi.org/10.1038/s41559-020-01321-y.
von Eugen, K., Endepols, H., Drzezga, A., Neumaier, B., Güntürkün, O., Backes, H. and Ströckens, F., 2022. Avian neurons consume three times less glucose than mammalian neurons. Current Biology [Online], 32(19), pp.4306–4313.e4. Available from: https://doi.org/10.1016/j.cub.2022.07.070.
Gavrilets, S. and Losos, J.B., 2009. Adaptive Radiation: Contrasting Theory with Data. Science [Online], 323(5915), pp.732–737. Available from: https://doi.org/10.1126/science.1157966.
Gould, S.J., 1966. Allometry and Size in Ontogeny and Phylogeny. Biological Reviews [Online], 41(4), pp.587–638. Available from: https://doi.org/10.1111/j.1469-185X.1966.tb01624.x.
Hallgrímsson, B., Willmore, K. and Hall, B.K., 2002. Canalization, developmental stability, and morphological integration in primate limbs. American Journal of Physical Anthropology [Online], 119(S35), pp.131–158. Available from: https://doi.org/10.1002/ajpa.10182.
Hamilton, M.B., 2009. Population genetics. Chichester, UK ; Hoboken, NJ: Wiley-Blackwell.
Hone, D.W.E. and Benton, M.J., 2005. The evolution of large size: how does Cope’s Rule work? Trends in Ecology & Evolution [Online], 20(1), pp.4–6. Available from: https://doi.org/10.1016/j.tree.2004.10.012.
Hone, D.W.E., Dyke, G.J., Haden, M. and Benton, M.J., 2008. Body size evolution in Mesozoic birds. Journal of Evolutionary Biology [Online], 21(2), pp.618–624. Available from: https://doi.org/10.1111/j.1420-9101.2007.01483.x.
Jetz, W., Thomas, G.H., Joy, J.B., Hartmann, K. and Mooers, A.O., 2012. The global diversity of birds in space and time. Nature [Online], 491(7424), pp.444–448. Available from: https://doi.org/10.1038/nature11631.
Kingsolver, J.G. and Pfennig, D.W., 2004. Individual-Level Selection as a Cause of Cope’s Rule of Phyletic Size Increase. Evolution [Online], 58(7), pp.1608–1612. Available from: https://doi.org/10.1111/j.0014-3820.2004.tb01740.x.
Kriegman, S., Cheney, N. and Bongard, J., 2018. How morphological development can guide evolution. Scientific Reports [Online], 8(1), p.13934. Available from: https://doi.org/10.1038/s41598-018-31868-7.
Ksepka, D.T., Balanoff, A.M., Smith, N.A., Bever, G.S., Bhullar, B.-A.S., Bourdon, E., Braun, E.L., Burleigh, J.G., Clarke, J.A., Colbert, M.W., Corfield, J.R., Degrange, F.J., De Pietri, V.L., Early, C.M., Field, D.J., Gignac, P.M., Gold, M.E.L., Kimball, R.T., Kawabe, S., Lefebvre, L.,
Marugán-Lobón, J., Mongle, C.S., Morhardt, A., Norell, M.A., Ridgely, R.C., Rothman, R.S.,
Scofield, R.P., Tambussi, C.P., Torres, C.R., van Tuinen, M., Walsh, S.A., Watanabe, A., Witmer, L.M., Wright, A.K., Zanno, L.E., Jarvis, E.D. and Smaers, J.B., 2020. Tempo and Pattern of Avian Brain Size Evolution. Current Biology [Online], 30(11), pp.2026–2036.e3. Available from: https://doi.org/10.1016/j.cub.2020.03.060.
Langston, W., 1981. Pterosaurs. Scientific American, 244(2), pp.122–137.
Liem, K.F. and Osse, J.W.M., 1975. Biological Versatility, Evolution, and Food Resource Exploitation in African Cichlid Fishes. American Zoologist [Online], 15(2), pp.427–454. Available from: https://doi.org/10.1093/icb/15.2.427.
Lyson, T.R., Bever, G.S., Bhullar, B.-A.S., Joyce, W.G. and Gauthier, J.A., 2010. Transitional fossils and the origin of turtles. Biology Letters [Online], 6(6), pp.830–833. Available from: https://doi.org/10.1098/rsbl.2010.0371.
Marugán-Lobón, J., Nebreda, S.M., Navalón, G. and Benson, R.B.J., 2022. Beyond the beak: Brain size and allometry in avian craniofacial evolution. Journal of Anatomy [Online], 240(2), pp.197–209. Available from: https://doi.org/10.1111/joa.13555.
Marugán-Lobón, J., Watanabe, A. and Kawabe, S., 2016. Studying avian encephalization with geometric morphometrics. Journal of Anatomy [Online], 229(2), pp.191–203. Available from: https://doi.org/10.1111/joa.12476.
McShea, D.W., 1994. Mechanisms of Large-Scale Evolutionary Trends. Evolution [Online], 48(6), pp.1747–1763. Available from: https://doi.org/10.2307/2410505.
Navalón, G., Bjarnason, A., Griffiths, E. and Benson, R.B.J., 2022. Environmental signal in the evolutionary diversification of bird skeletons. Nature [Online], 611(7935), pp.306–311. Available from: https://doi.org/10.1038/s41586-022-05372-y.
Olkowicz, S., Kocourek, M., Lučan, R.K., Porteš, M., Fitch, W.T., Herculano-Houzel, S. and Němec, P., 2016. Birds have primate-like numbers of neurons in the forebrain. Proceedings of the National Academy of Sciences [Online], 113(26), pp.7255–7260. Available from: https://doi.org/10.1073/pnas.1517131113.
Olson, E.C. and Miller, R.L., 1999. Morphological Integration [Online]. Chicago, IL: University of Chicago Press. Available from: https://press.uchicago.edu/ucp/books/book/chicago/M/bo3620375.html [Accessed 13 January 2023].
Pigot, A.L., Sheard, C., Miller, E.T., Bregman, T.P., Freeman, B.G., Roll, U., Seddon, N., Trisos, C.H., Weeks, B.C. and Tobias, J.A., 2020. Macroevolutionary convergence connects morphological form to ecological function in birds. Nature Ecology & Evolution [Online], 4(2), pp.230–239. Available from: https://doi.org/10.1038/s41559-019-1070-4.
Salazar-Ciudad, I., 2007. On the origins of morphological variation, canalization, robustness, and evolvability. Integrative and Comparative Biology [Online], 47(3), pp.390–400. Available from: https://doi.org/10.1093/icb/icm075.
Scharloo, W., 1991. Canalization: Genetic and Developmental Aspects. Annual Review of Ecology and Systematics, 22, pp.65–93.
Simpson, G.G., 1944. Tempo and Mode in Evolution [Online]. Columbia University Press. Available from: https://www.degruyter.com/document/doi/10.7312/simp93040/html [Accessed 13 January 2023].
Stanley, S.M., 1973. An Explanation for Cope’s Rule. Evolution [Online], 27(1), pp.1–26. Available from: https://doi.org/10.2307/2407115.
Tsuboi, M., van der Bijl, W., Kopperud, B.T., Erritzøe, J., Voje, K.L., Kotrschal, A., Yopak, K.E., Collin, S.P., Iwaniuk, A.N. and Kolm, N., 2018. Breakdown of brain–body allometry and the encephalization of birds and mammals. Nature Ecology & Evolution [Online], 2(9), pp.1492–1500. Available from: https://doi.org/10.1038/s41559-018-0632-1.
Waddington, C.H., 1953. Genetic Assimilation of an Acquired Character. Evolution [Online], 7(2), pp.118–126. Available from: https://doi.org/10.2307/2405747.
Wills, M.A., Briggs, D.E.G. and Fortey, R.A., 1994. Disparity as an evolutionary index: a comparison of Cambrian and Recent arthropods. Paleobiology [Online], 20(2), pp.93–130. Available from: https://doi.org/10.1017/S009483730001263X.
Xu, X., You, H., Du, K. and Han, F., 2011. An Archaeopteryx-like theropod from China and the origin of Avialae. Nature [Online], 475(7357), pp.465–470. Available from: https://doi.org/10.1038/nature10288.
Yalden, D.W., 1984. What size was Archaeopteryx? Zoological Journal of the Linnean Society [Online], 82(1–2), pp.177–188. Available from: https://doi.org/10.1111/j.1096-3642.1984.tb00541.x.
Comments